我们使用 cookie 来使你的体验更好。 为了遵守新的 e-隐私指令,我们需要问您同意设置的 cookie。
Solvent Choice for Microwave Synthesis
The more efficient a solvent is in coupling with the microwave energy, the faster the temperature of the reaction mixture increases.
Solvents play a very important role in organic synthesis. Most reactions take place in solution, and therefore, choice of solvent can be a crucial factor in the outcome of a reaction. One of the most important characteristics of a solvent is its polarity. With microwave heating, this becomes a more significant component, as microwaves directly couple with the molecules that are present in the reaction mixture. The more polar a reaction mixture is, the greater its ability to couple with the microwave energy. As discussed in the previous chapter, this interaction leads to a rapid rise in temperature and faster reaction rates. This chapter will discuss the theory behind solvent polarity and how it pertains to the individual solvents, their physical constants, and how they behave in a microwave field. In addition, the last section will discuss how to choose a solvent in a microwave-enhanced organic reaction.
How microwaves heat solvent
Many factors characterize the polarity of a solvent. Intrinsically, the dielectric constant, dipole moment, dielectric loss, tangent delta, and dielectric relaxation time all contribute to an individual solvent’s absorbing characteristics. The dielectric constant (ε), also known as the relative permittivity, of a solvent measures its ability to store electric charges. Mathematically, it is the ratio of the electrical capacity of a capacitor filled with the solvent to the electrical capacity of the evacuated capacitor (ε=Cfilled/Cevacuated). This value, when measured, is dependent on both temperature and frequency.
The dipole moment, which is measured in Debye units (D), is also a mathematical entity. It is the product of the distance between the centers of charge in the solvent molecule multiplied by the magnitude of that charge. One equation used to determine dipole moment is: T = pE (T = torque, p = dipole moment, and E = field strength). The magnitude can also be defined as: μ = Qr (μ = dipole moment, Q = charge, and r = distance between charges). Molecules with large dipole moments also have large dielectric constants. This is because polarization depends on dipole rotation — the ability of a molecule’s dipole to align with a rapidly changing electric field.17
The ability of a substance to convert electromagnetic energy into heat at a given frequency and temperature is determined by the following equation: tan δ = εʺ/ε. Tangent delta (δ), or loss tangent, is the dissipation factor of the sample or how efficiently microwave energy is converted into thermal energy. It is defined as the ratio of the dielectric loss, or complexed permittivity (εʺ), to the dielectric constant (ε). Dielectric loss is the amount of input microwave energy that is lost to the sample by being dissipated as heat. It is this value, εʺ, that best provides the organic chemist with the coupling efficiency of a particular solvent. This will be discussed in greater detail in the following section.
Loss Tangent
Equation
tan δ = εʺ/ε
The three main dielectric parameters, tangent delta, dielectric constant, and dielectric loss, are all related to the ability of a solvent to absorb microwave energy. Molecular relaxation time has a large effect on these parameters. The dielectric relaxation time is the time it takes a molecule to achieve 63% of its return to randomized disorder from an organized state after an applied microwave field is removed.1 Functional groups, temperature, frequency, and volume will all influence the relaxation time of a solvent. Most commercial microwave systems are set to a frequency of 2450 MHz. At this frequency, the only thing that can change the three parameters is temperature. As the temperature of a solvent increases, a decrease in its relaxation time and dielectric parameters will be seen, and hence, its coupling efficiency. There are a few exceptions, but this is generally the trend. A graphical representation of this effect for the tangent delta, dielectric constant, and dielectric loss values of 17 common solvents is shown in Figures 10-12, respectively.
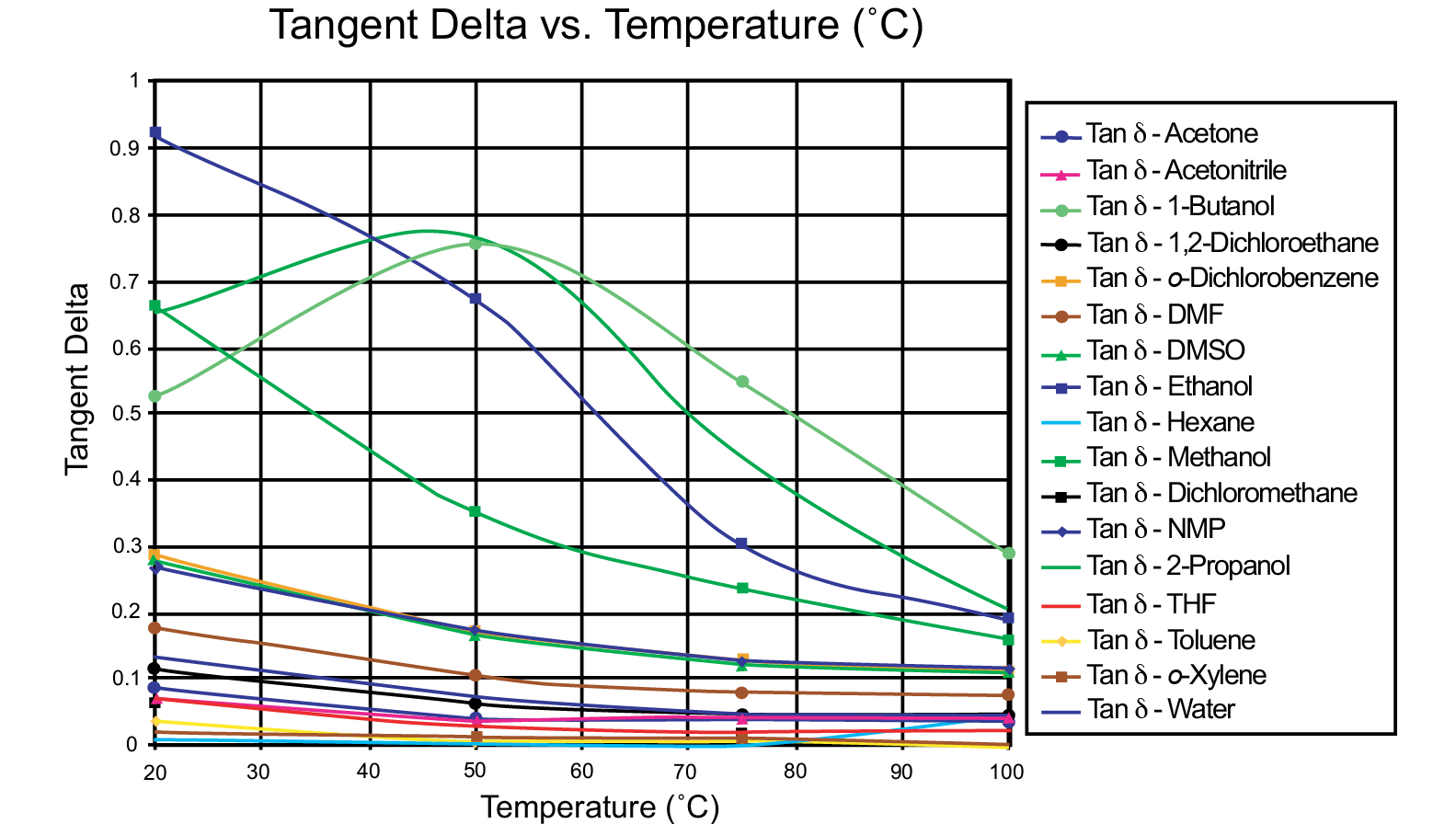
Figure 10: Tangent Delta versus Temperature
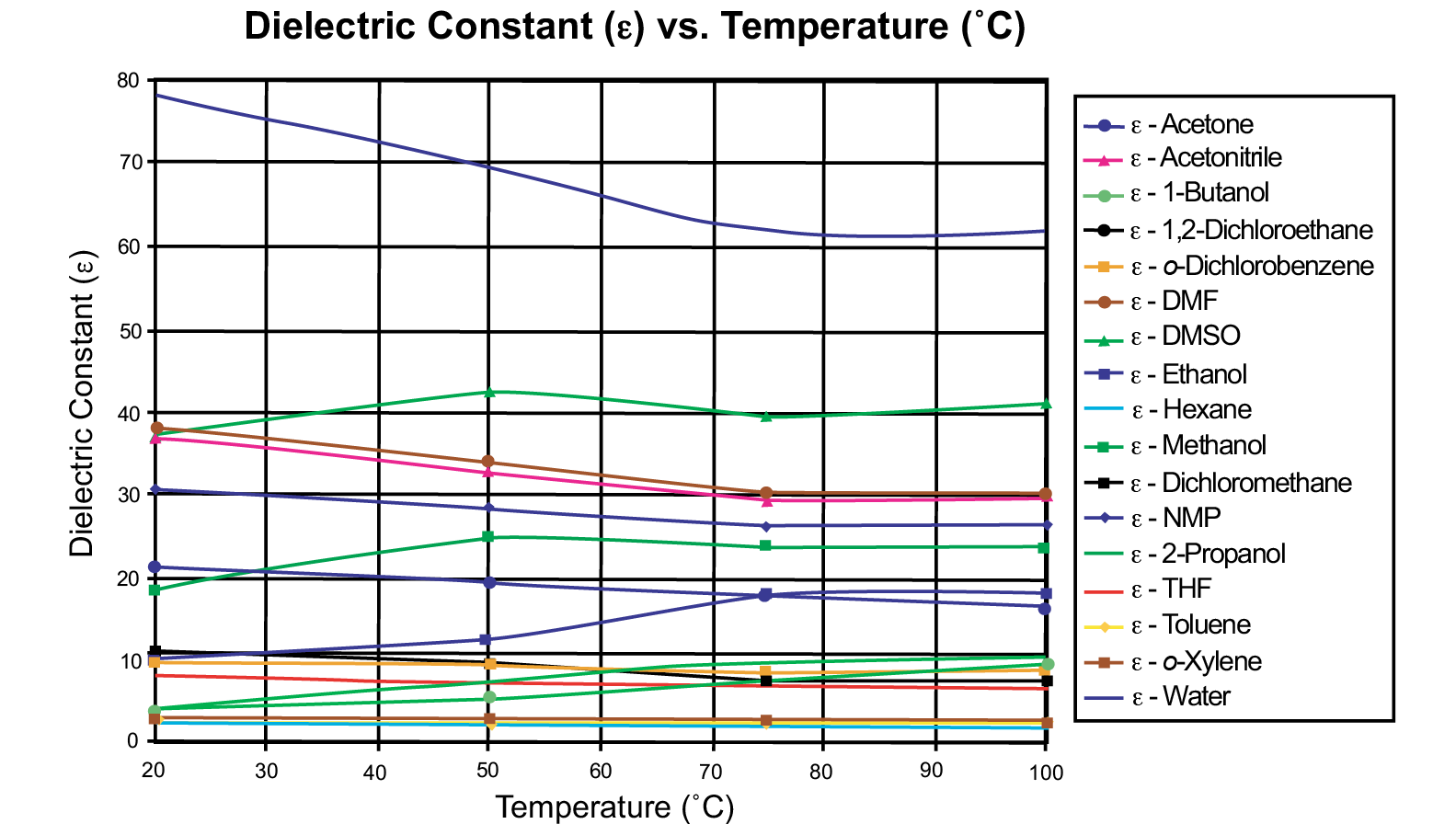
Figure 11: Dielectric Constant versus Temperature
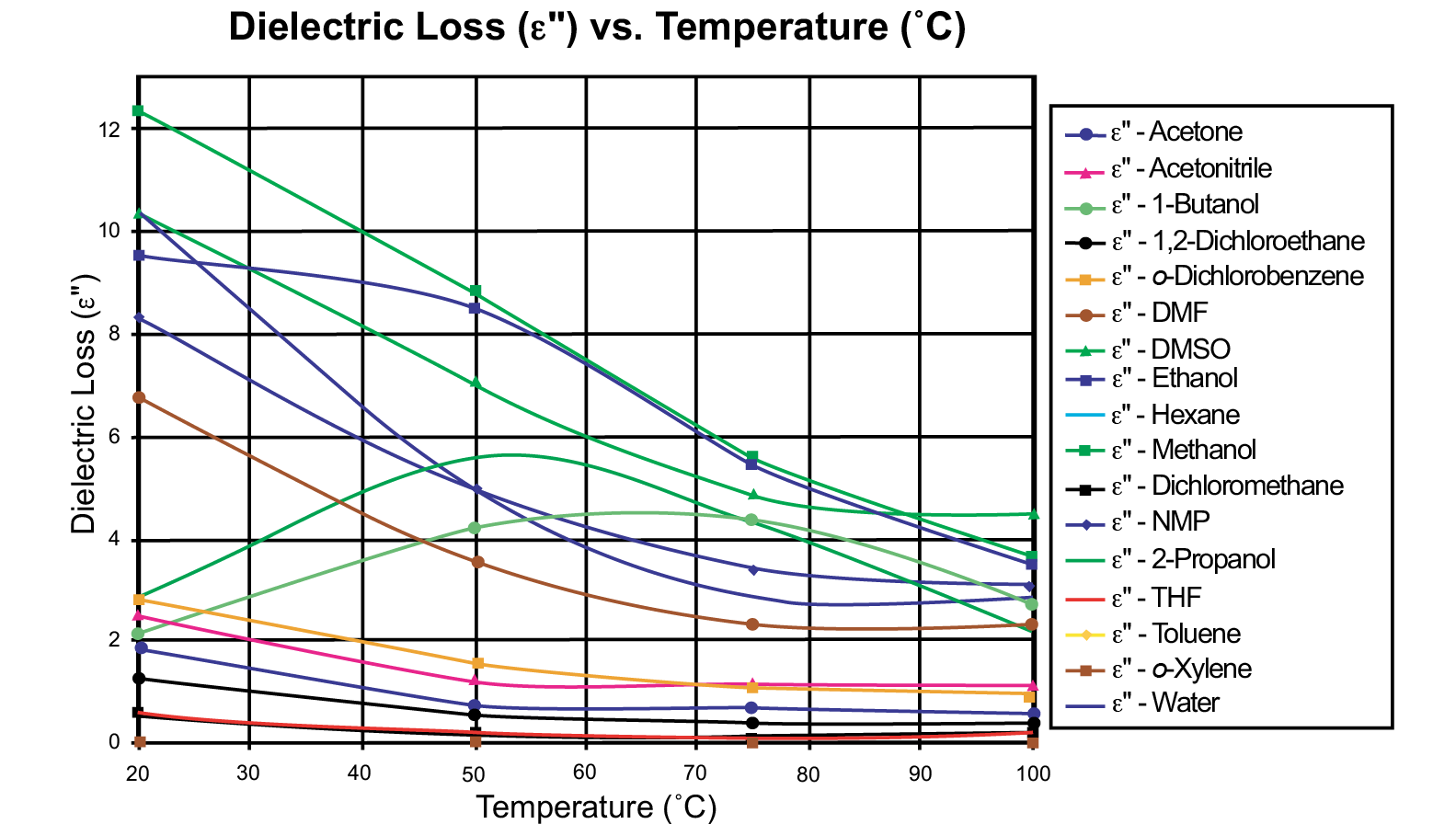
Figure 12: Dielectric Loss versus Temperature
Organic Solvents for Microwave Synthesis
When considering solvents for a microwave-enhanced organic reaction, a chemist must now realize that boiling points become a less important factor in that decision. Microwave energy (300 W) will reach and bypass the boiling point of most solvents in a matter of seconds. Using pressurized reaction vessels provide for greater use of the lower boiling point solvents that are normally ignored in conventional high temperature reactions. Alternatively, one factor that becomes more important is how efficient the molecules in a solvent or a solvent mixture couple with an applied microwave field.
Microwave energy will reach and bypass the boiling point of most solvents in a matter of seconds.
As we established in the previous section, the three main dielectric parameters all factor into the ability of a solvent to absorb microwave energy, but they do so quite differently. Table 1 has been developed to show this difference in thirty common solvents. There are three main columns (dielectric constant, tan δ, and dielectric loss, respectively), which are indicated by the bold lines. The data, which was measured at room temperature and at a frequency of 2450 MHz, is shown in descending order.17,18a We find that the values, and their respective solvents, represented in the third column (dielectric loss) are most indicative of how quickly a solvent will reach its desired temperature. In general, the higher the number, the more efficient the solvent converts microwave energy into thermal energy, and thus, the faster the temperature will increase.
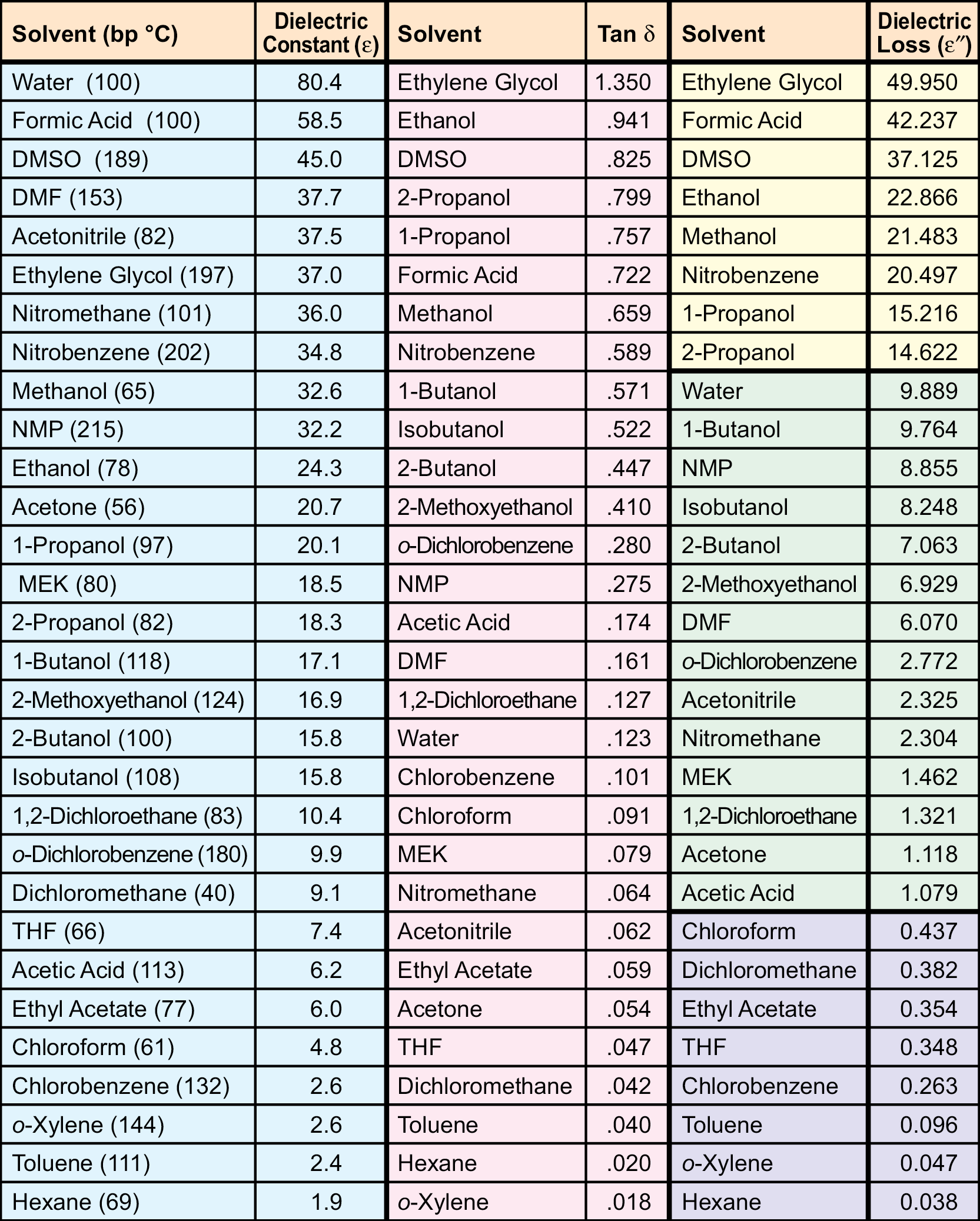
Table 1: Dielectric constant (ε), tan δ, and dielectric loss (εʺ) for 30 common solvents (measured at room temperature and 2450 MHz)
The solvents in Table 1 can easily be categorized into three different groups: high, medium, and low absorbing solvents. By examining the dielectric loss values from the third column of the table, one can see where significant gaps in between the numbers are present (bold lines). The high absorbing solvents are ones that have dielectric losses greater than 14.00. Medium absorbers would generally have dielectric loss values between 1.00 and 13.99, and low absorbing molecules have dielectric losses that are less than 1.00. High absorbers like small chain alcohols, dimethyl sulfoxide (DMSO), and nitrobenzene all have large dielectric losses, so they heat very quickly within the microwave chamber. Common organic solvents that are grouped as medium absorbers include dimethylformamide (DMF), acetonitrile, butanols, ketones, and water. These, too, heat very efficiently, but they require more time to reach desired temperatures. Additionally, chloroform, dichloromethane, ethyl acetate, and, as expected, ethers and hydrocarbons, are very low microwave absorbing solvents. They can be heated to temperatures well above their boiling points, but they take much longer.
Water, for example, has the highest dielectric constant (80.4) of the thirty solvents, but its tangent delta and dielectric loss values do not rank at the top of their respective lists. If we only considered the dielectric constant, we would assume that water is the most polar solvent in a microwave field. This is not the case. It should be classified as a medium absorber, which is where the second and third columns (tan δ and dielectric loss) categorize it. In another example, acetonitrile is ranked fairly high in the dielectric constant column with a value of 37.5. Looking at the tangent delta values, acetonitrile plummets close to the bottom at 0.062. So, what kind of solvent is acetonitrile? Once again, we look to the third column where acetonitrile falls in the middle with a dielectric loss value of 2.325. Acetonitrile should be considered as a medium absorber.
In addition to the coupling efficiency of a solvent, a chemist should also be aware of the pressures that are generated at certain temperatures in a sealed tube for that solvent. A pressurized environment can be very advantageous to many different kinds of chemistries. As the temperature of a solvent increases above its boiling point, more and more pressure builds up in the reaction vessel. In solvent-only experiments, the pressure that is generated for a specific temperature is independent of the solvent volume to head space ratio. (The head space is the volume of a 10-mL capacity pressure tube that is not occupied by the solvent at room temperature.) This will not be true for actual chemical reactions, as newly formed molecules are constantly being introduced into the gas phase throughout the duration of the reaction.
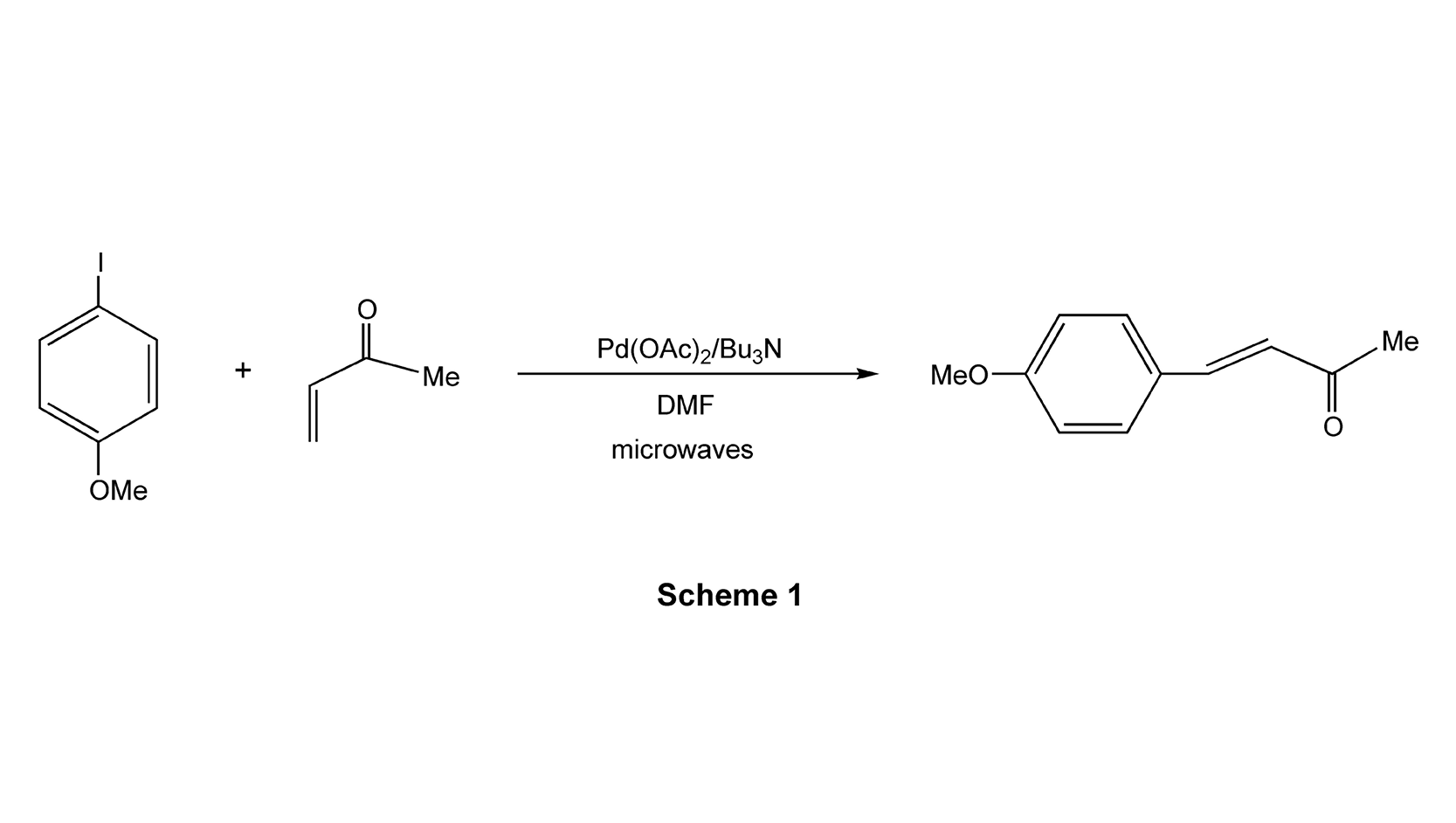
Scheme 1
Water becomes a more interesting solvent at higher temperatures and pressures. Under normal conditions, water maintains a very high dielectric constant and persistent hydrogen bonding. As the temperature and pressure of water increases, it begins to act more like an organic solvent. It changes from a very polar liquid to an almost nonpolar one and organic compounds become more soluble. With these enhanced conditions, water has increased acidity, reduced density, and a lower dielectric constant. With microwaves, the supercritical levels of water (Tc = 374 °C, Pc = 218 atm = 3204 psi = 221 bar), where gaseous and liquid water coexist, are not quite reached. Nevertheless, the increased temperatures and pressures can be advantageous for organic synthesis in aqueous media.
Many solvents decompose to hazardous components from prolonged exposure to high temperatures. Prior to choosing an organic solvent, a chemist should be aware of the stability of that solvent at high temperatures. This information is provided in Section 10 (Stability and Reactivity) of the Material Safety Data Sheet (MSDS) for that particular solvent. For example, a few of the more commonly used solvents can degenerate to hazardous components at high temperatures. Dichloromethane, 1,2-dichloroethane, and chloroform are among the chlorine-containing solvents and will decompose to hydrochloric acid (HCl), carbon monoxide (CO), and carbon dioxide (CO2). In addition, both dichloromethane and chloroform will also yield the highly toxic phosgene (ClCOCl). Dimethylformamide (DMF), dimethylacetamide (DMA), acetonitrile, triethylamine, pyridine, and N-methyl-pyrrolidinone (NMP) all will decompose to carbon monoxide (CO), carbon dioxide (CO2), and nitrogen oxides (NxOy). It should be noted that if DMF becomes discolored, it may cause vessel failures and release toxic fumes. Additionally, pyridine and acetonitrile can produce cyanides. Dimethyl sulfoxide (DMSO) also decomposes to toxic components at high temperatures. It can yield sulfur dioxide (SO2), formaldehyde (CH2O), methyl mercaptan (MeSH), dimethyl sulfide (Me2S), dimethyl disulfide (Me2S2), and bis(methylthio)methane (CH2(SMe)2). Upon exposure to high temperatures, hexamethylphosphoramide (HMPA) will turn a cloudy yellow-orange. Thermal decomposition of HMPA produces toxic fumes of phosphines and phosphorous oxides. These are just a few safety issues that an organic chemist should be aware of when performing high temperature reactions in pressurized vessels.
Ionic liquids
Ionic liquids are becoming promising and useful substitutes for standard organic solvents. Not only are they environmentally benign, but they also possess unique chemical and physical properties.19 As their name indicates, ionic liquids are only comprised of ions and can also be referred to as fused salts. In general, these fused salts contain one positively charged ion and one negatively charged ion. They have a vast liquid temperature range of almost 300 °C, from –96 °C to 200 °C (unlike water which only has a range of 100 °C). Though they usually consist of poorly coordinating ions, ionic liquids are highly polar, nonvolatile, and readily dissolve both organic and inorganic compounds. These characteristics are all quite beneficial to synthetic organic chemists.
Ionic liquids are either organic salts or mixtures consisting of at least one organic component. They are usually prepared by metathesis of a halide salt of the desired cation with a Group 1 metal or an ammonium salt of the desired anion. Figure 39 shows the most common salts, which are alkylammonium, alkylphosphonium, N-alkylpyridinium, and N,N-dialkylimidazolium cations, respectively. The anion may be organic or inorganic, and there are a number of options to choose from: CH3COO-, CF3COO-, F-, Cl-, Br-, I-, BF4-, PF6-, NO3-, AlCl4-, FeCl4-, NiCl3-, ZnCl3-, and SnCl5-. Figure 40 exhibits common ionic liquids, some of which are even commercially available.

Figure 39: Common ionic liquid cations
Ionic liquids, also known as fused salts, contain one positively charged ion and one negatively charged ion.
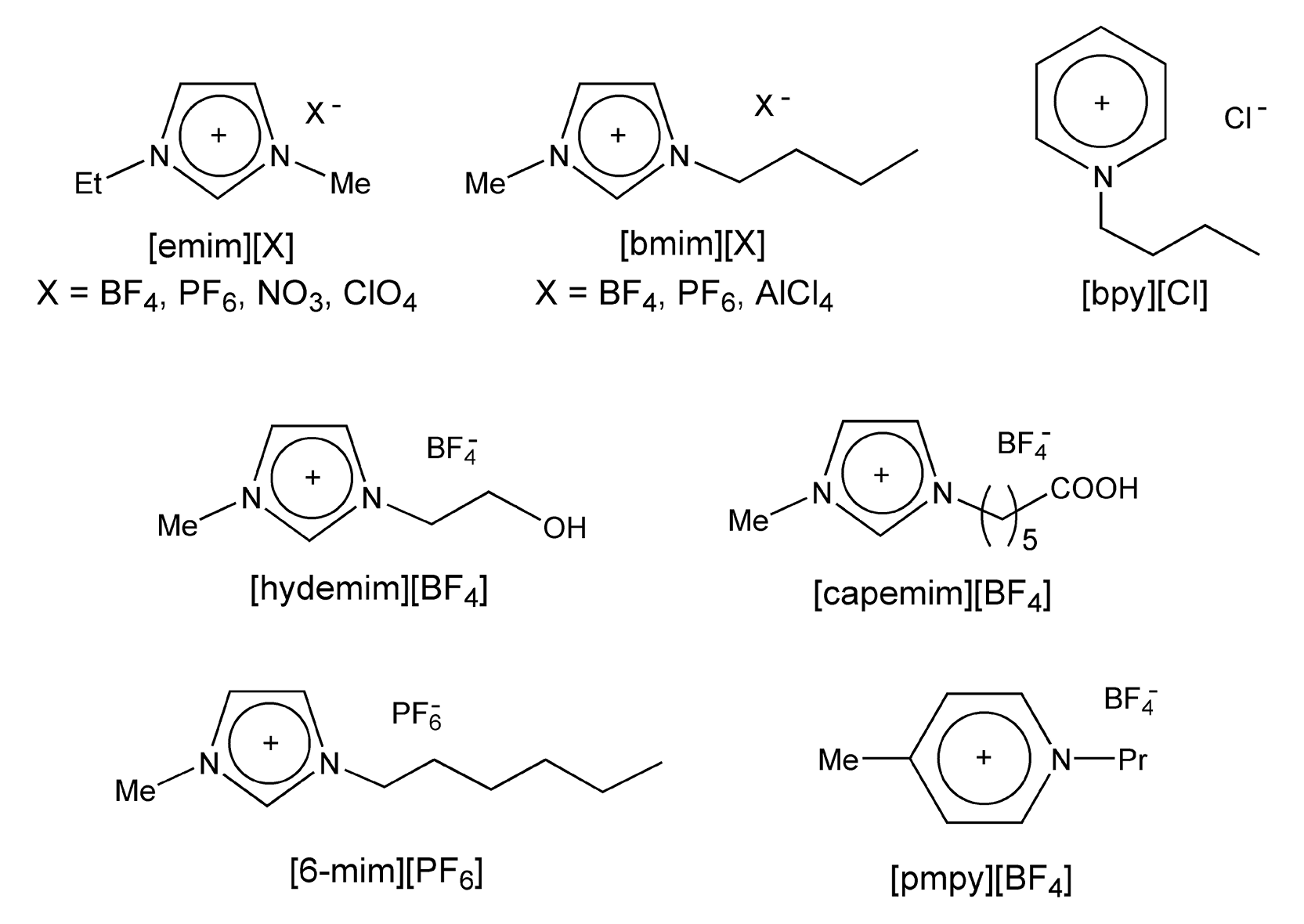
Figure 40: Common ionic liquids
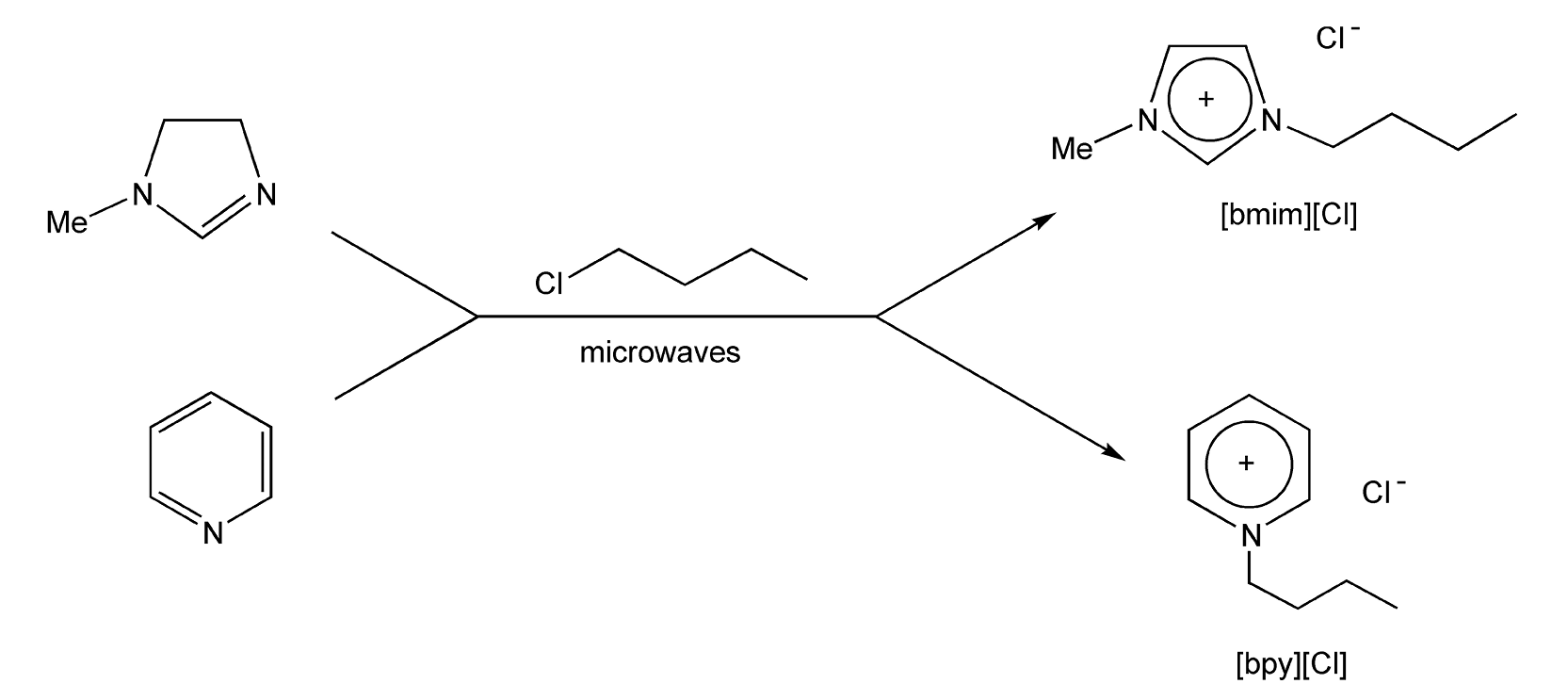
Scheme 2
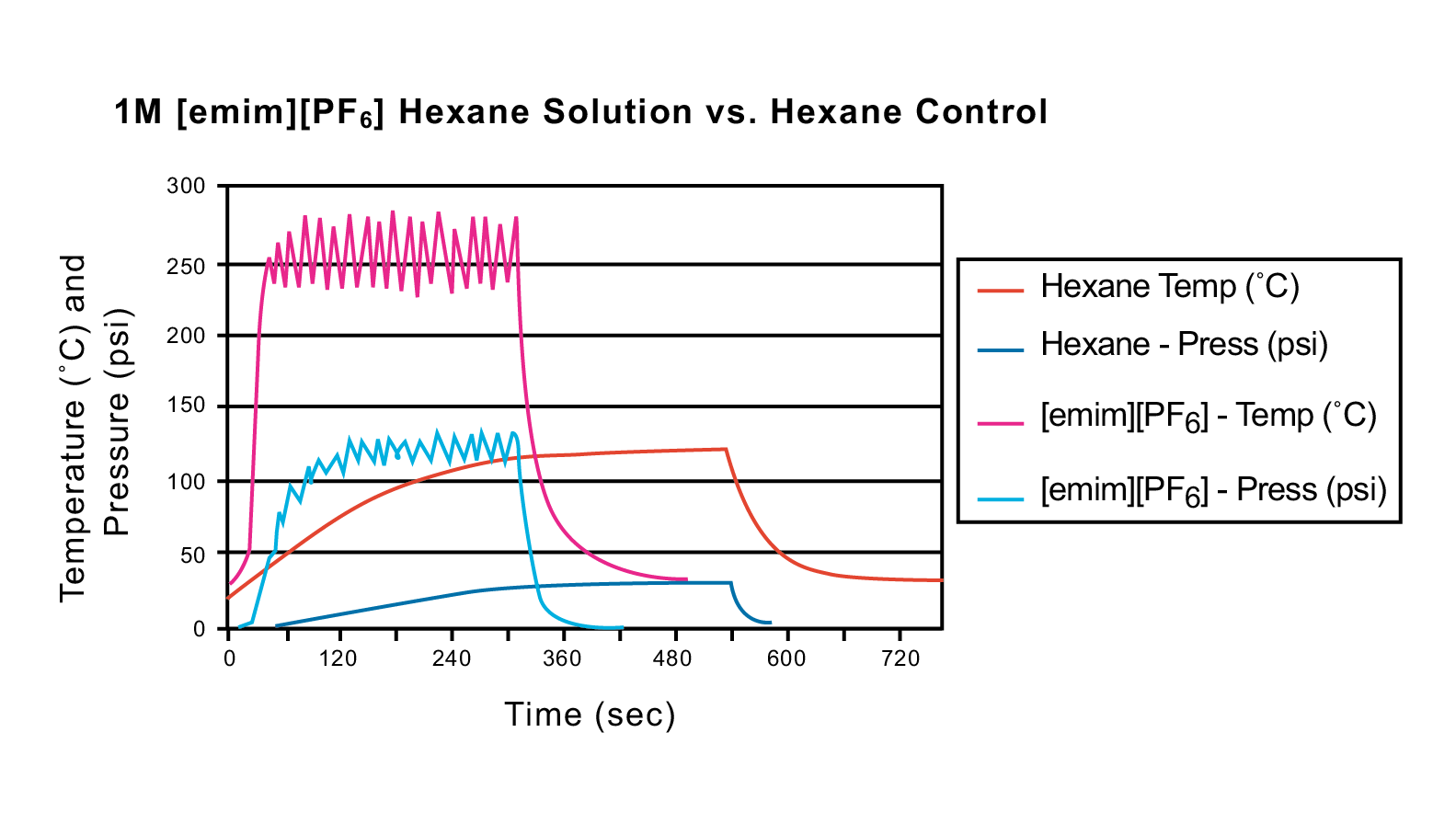
Figure 41: Temperature and pressure curve comparison between a 1M [emim][PF6] hexane solution (2 mL) and a 2-mL hexane control
The conventional preparation of ionic liquids is quite time consuming, as they can require up to 7 days of reflux; thus, microwave irradiation is a preferable method to activate and speed up ionic liquid synthesis.20,21 Khadilkar et al. synthesized both 1-butyl-3-methylimid-azoliumchloride [bmim][Cl] and 1-butylpyridiniumchloride [bpy][Cl] in 60 minutes and 22 minutes, respectively, with microwave heating (Scheme 2).21
Microwave irradiation has also been used to enhance organic reactions in which an ionic liquid is used as the solvent. As discussed in the introduction, the two fundamental mechanisms for transferring energy from microwaves to the substance being heated are either dipole rotation or ionic conduction. Ionic liquids absorb microwave irradiation extremely well and transfer energy rapidly by ionic conduction. Figures 41 and 42 exhibit the temperature and pressure curves for two ionic liquids in 2 mL of hexane (1M [emim][PF6] and 1M [emim][BF4], respectively) versus a 2-mL hexane control. Each sample was run with the following programmed method: 100 W, 5 min ramp time, 5 min hold time, 250 °C, 250 psi. All runs were performed in 10-mL pressure tubes. As the graphs indicate, the hexane control did not reach the maximum set temperature, and it needed nine minutes to even reach 120 °C. In both 1M ionic liquid solutions, the temperature was met and exceeded in less than 40 seconds. Additional ionic liquid experimentation in solvents has recently been published by Leadbeater et al.22
The following 1,3-dipolar cycloaddition is an example of a microwave-enhanced organic reaction in an ionic liquid (Scheme 3).23 Scheme 4 shows a microwave-induced Knoevenagel condensation reaction between a malonate derivative (EWG = electron withdrawing group) and a grafted ionic liquid phase.24,25 After the reaction occurs, the ionic liquid phase can be removed and then regenerated for future use.
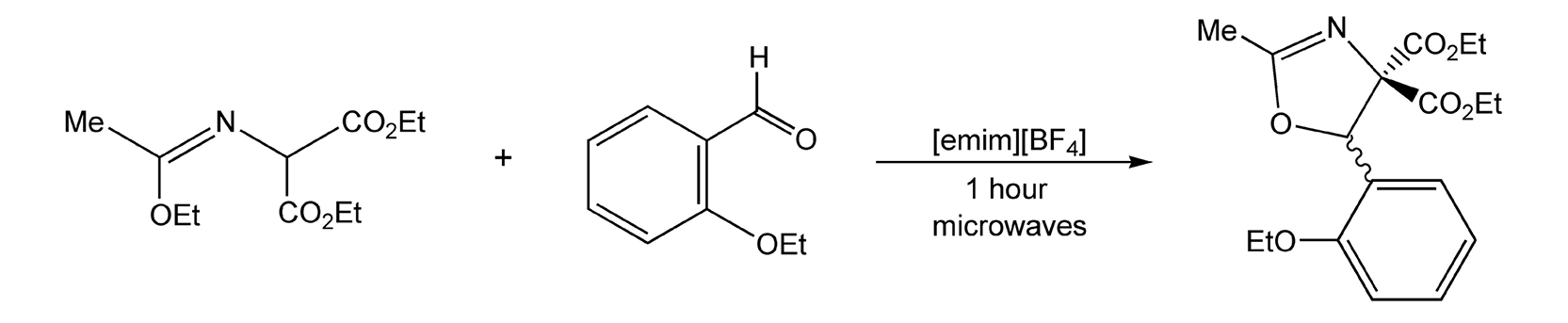
Scheme 3
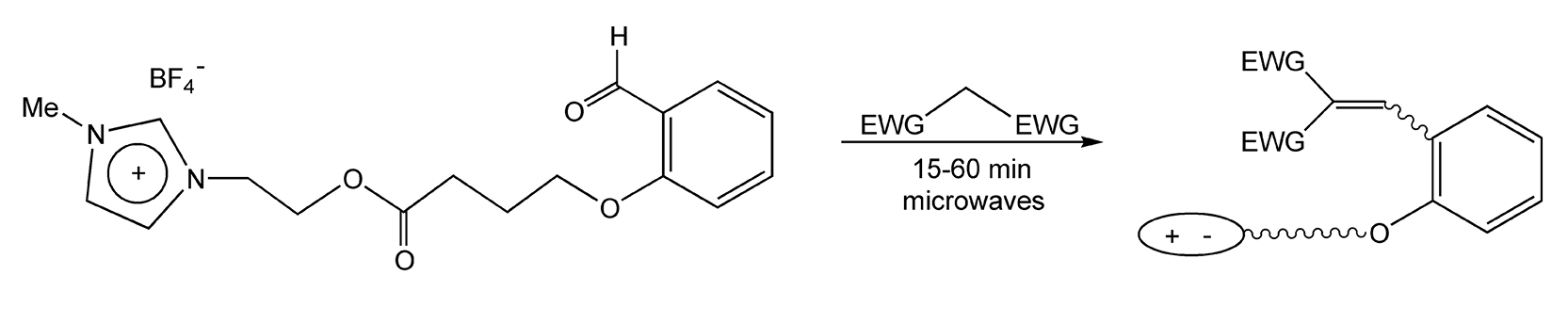
Scheme 4
Choosing a solvent
An important step before attempting a microwave enhanced organic reaction is choosing a solvent. As discussed earlier in this chapter, the coupling efficiency of a solvent is very important to the outcome of the reaction. The more efficient a solvent is in coupling with the microwave energy, the faster the temperature of the reaction mixture increases. Table 27 contains some common organic solvents that have been categorized as high, medium, or low absorbers. It is a condensed version of Table 1. In addition, a pressurized environment can be very advantageous in performing many different kinds of chemistries. Microwave energy (300 W) will reach and bypass the boiling point (Tables 2-26) of most solvents in a matter of seconds. Using pressurized reaction vessels provide for greater use of the lower boiling point solvents that are normally ignored in conventional high temperature reactions.
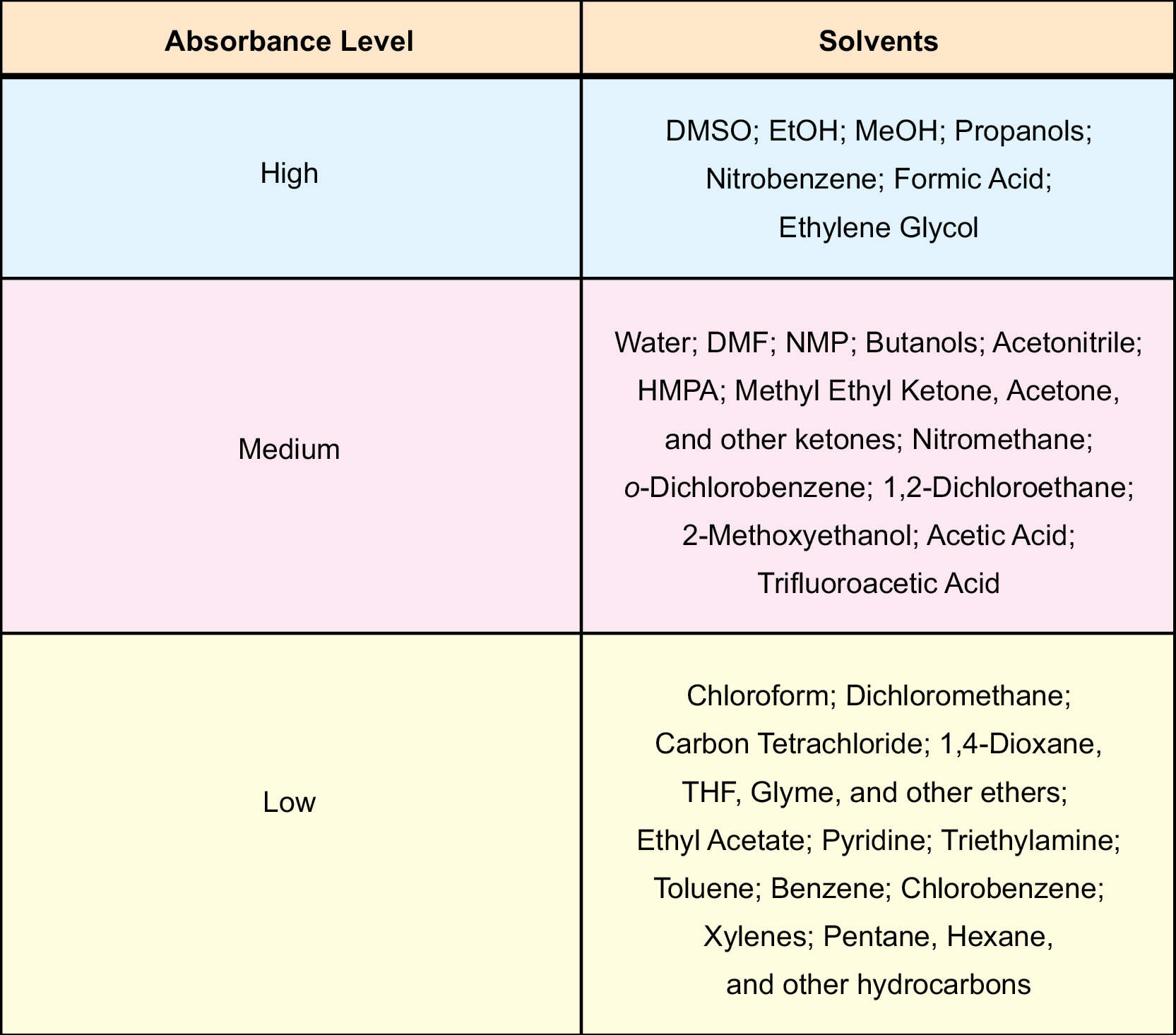
Table 27: High, Medium, and Low absorbing solvents
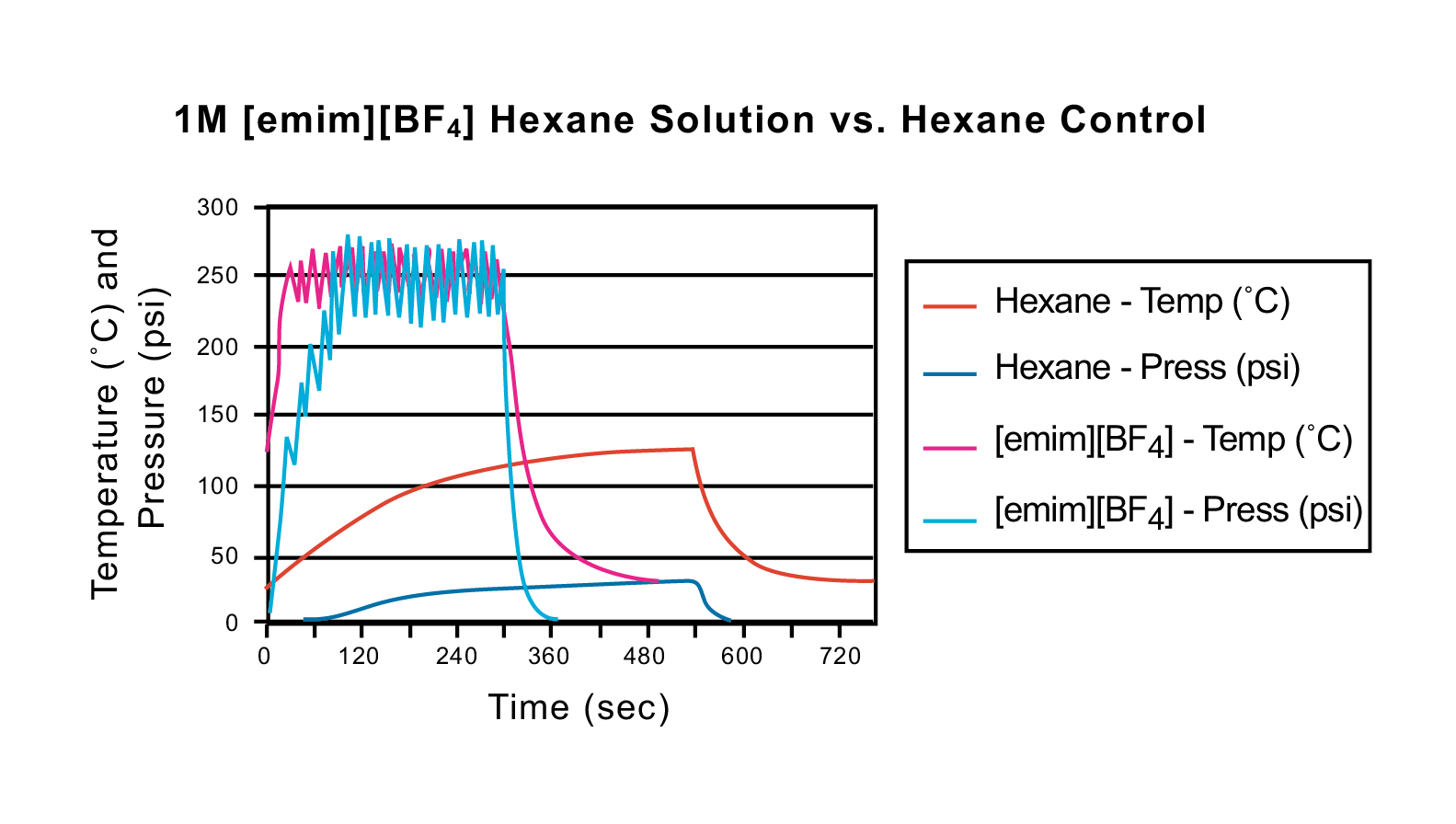
Figure 42: Temperature and pressure curve comparison between a 1M [emim][BF4] hexane solution (2 mL) and a 2-mL hexane control
Choosing a solvent can be a dilemma. The first question to ask is whether high temperature, high pressure, or high energy is needed. When using conventional heat, chemists are usually concerned with the temperature and select a solvent accordingly. Pressure vessels and oil baths are used with higher boiling point solvents to maximize the temperature increase. Achieving high temperatures with microwaves is not the task here; they can do that with hardly any effort. If a high temperature is required, then choose a solvent that will reach the set temperature. If maintaining a high pressure is all that is desired, then set a high maximum temperature for a low boiling point solvent. The pressure will increase rapidly as the temperature continues to rise above its boiling point. These are classical requirements that microwave energy can easily provide.
The more efficient a solvent is in coupling with the microwave energy, the faster the temperature of the reaction mixture increases.
High energy is different. It is why microwaves have produced dramatically favorable results and the reason microwave energy is so beneficial to organic synthesis, as opposed to conventional heating which is much slower. The energy transfer in a microwave-assisted reaction is incredibly quick: energy is transferred every nanosecond that it is applied. When performing a microwave reaction, the user can program the power, temperature, time, and, in some cases, a pressure limit. As the temperature reaches the input value, the power is reduced so that the reaction mixture does not bypass the set point. It then stays at a lower level in order to maintain the set temperature throughout the entire reaction. It is the power, or energy, that is the most important variable in a microwave-enhanced reaction. Recent experimentation has shown that simultaneous cooling of the reaction vessel during a reaction will ensure a constant, high power level for direct molecular heating. This has dramatically affected reaction rates and nearly doubled the percent yields of some lower yielding reactions.18
One would assume that nonpolar solvents (i.e. hexane, benzene, toluene) are generally not used in microwave-assisted organic reactions. In Table 1, these solvents are all at the bottom of the three columns, possessing very low dielectric constants, tan δ values, and dielectric loss values. They do not couple very efficiently to microwave irradiation, and hence, would not heat a reaction very well. Conversely, a nonpolar solvent can act as a heat sink. Reaction mixtures that are temperature sensitive will benefit greatly from this capability. As microwaves are being added to a reaction, the nonpolar solvent, which is not interacting with the irradiation, will help to draw away the thermal heat being produced from the polar reagents. The reaction is still receiving activation energy, but its internal temperature will remain low. Simultaneous cooling of the microwave cavity can benefit this reaction condition and ensure a constant, high power level.
General synthetic organic chemistry rules still apply with microwave-assisted chemical reactions. Regardless of whether one is performing a nucleophilic substitution, electrophilic substitution, or elimination reaction, the type of solvent for each remains the same. There are protic and aprotic solvents, and each of these may or may not be applicable for certain kinds of chemistry. Protic solvents have the ability to solvate or interact with both cations and anions, whereas aprotics can only solvate cations. The solvents of each type are interspersed throughout Table 1. Chemists must use a combination of the previously discussed information from this chapter in order to determine their reaction conditions.
Nucleophilic substitution reactions (SN2, SN1, etc.) depend considerably on solvent effects for their success. Whether a transition state is stable or unstable in a solvent greatly influences the outcome of the reaction. Additionally, for SN2 reactions, stabilization or destabilization of the reactant nucleophile is also a major factor. As illustrated in Scheme 5, SN2 reactions can be categorized into four types (I, II, III, and IV), and they generally require aprotics (hexane, benzene, Et2O, CHCl3, ethyl acetate, acetone, HMPA, DMF, DMSO, acetonitrile).26 Protic solvents are disfavored in these reactions, since the ground state energy level of the attacking nucleophile is lowered by solvation. In other words, the nucleophile is stabilized by solvation and, therefore, less reactive towards the electrophile. The polarity of the aprotic solvent is also important. The only type in Scheme 5 that succeeds in highly polar solvents is Type II because the reactants are uncharged. The reactions of Types I, III, and IV have at least one charged reactant, and the reaction is actually hindered by a very polar solvent.
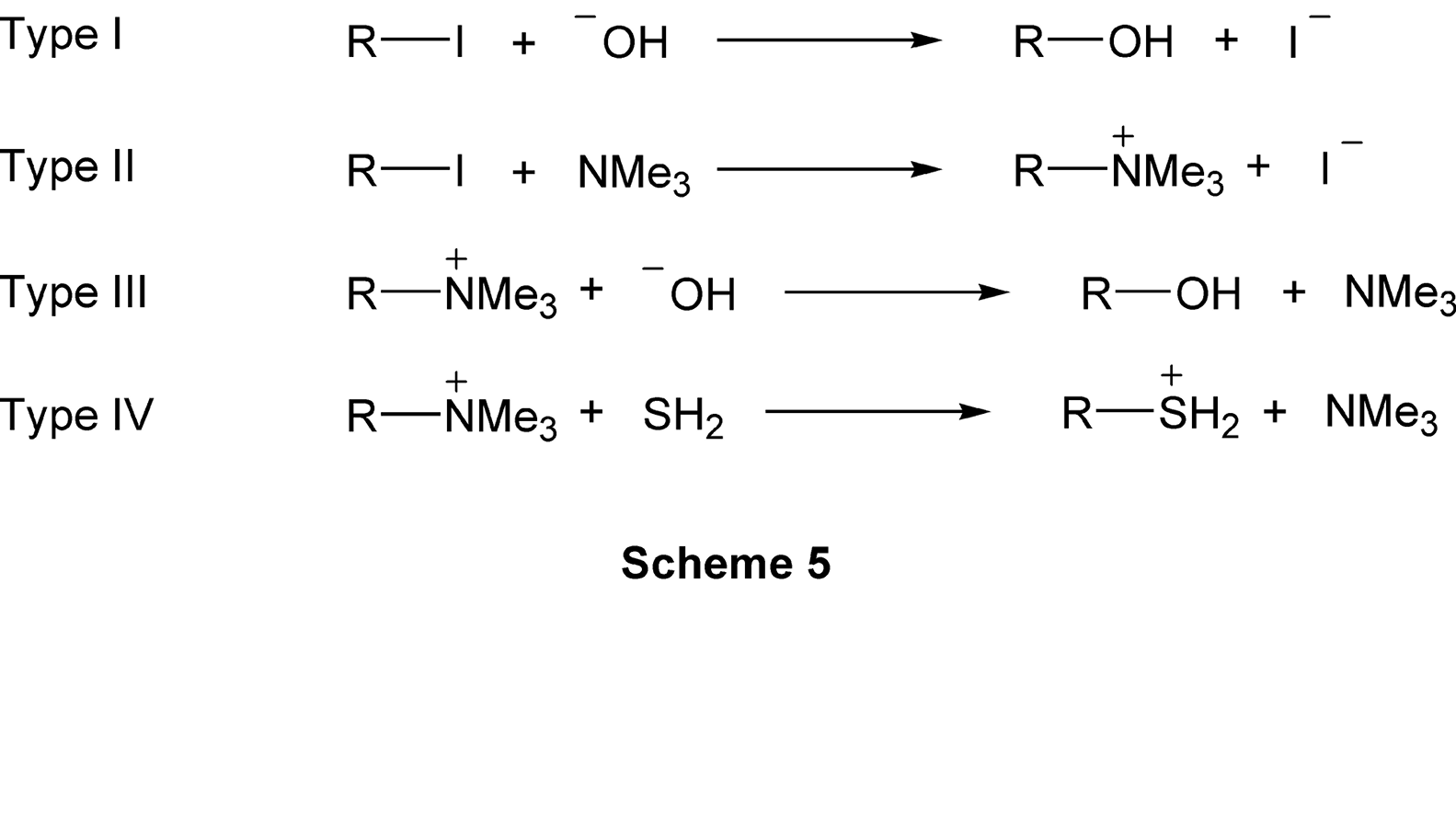
Scheme 5
Protic solvents solvate or interact with both cations and anions. Aprotic solvents only interact with cations.
The stability of the transition state in SN1 reactions is extremely important. The solvent used strongly influences this stability. SN1 reactions generally occur more rapidly in highly polar protic solvents than in aprotic and nonpolar solvents, though there are exceptions. The energy level of the transition state that leads to the carbocation intermediate is lowered by solvation. Imagine solvent molecules orienting themselves around the cation in such a manner that the electron-rich ends of the solvent dipoles face the positive charge. This is described in the Hammond postulate, which states that any factor stabilizing the intermediate carbocation should increase the rate of reaction.26 Alcohols, water, and formic acid are good solvents for SN1, but HMPA, DMF, and DMSO have also been known to work well since they are polar.
The solvent effects necessary for successful electrophilic substitution reactions are slightly different from those just discussed for nucleophilic substitutions. Mechanistically, SE2 is analogous to SN2 where a new bond forms as the old one breaks. One difference is in the solvent preference. The reaction rate increases as solvent polarity increases. The mechanism of the SE1 reaction is analogous to the SN1 reaction: slow ionization occurs as the bond breaks, followed by new bond formation. As with the SN1 reaction, SE1 reactions are faster and more successful in highly polar solvents.
Elimination reactions (a double bond is formed by either simultaneous (E2) or sequential (E1) leaving group departure and proton abstraction) are analogous to nucleophilic substitutions, E2 to SN2 and E1 to SN1. Interestingly, in many cases, an E2 reaction will compete with an SN2 in the same reaction mixture. Both contain a nucleophile that will happily abstract a proton or attack an electrophilic carbon. In general, increasing the polarity of the solvent will favor substitution over elimination. Alternatively, elimination will be more favorable if the solvent is nonionizing and in the presence of a strong base. For E1 reactions, a more polar solvent will enhance the rate of the mechanism, especially one that involves an ionic intermediate, as has been the case with the other two-step reaction mechanisms (SN1, SE1).
Thus, solvents play an extremely significant role in microwave-enhanced organic chemistry. Using the dielectric loss values from Table 1, coupled with the general rules of organic chemistry previously described in this chapter, chemists can now develop the specific conditions that will optimize their synthetic endeavors.
Instruments
1. Neas, E.D.; Collins, M.J. Introduction to Microwave Sample Preparation Theory and Practice, Kingston, H.M.; Jassie, L.B., Eds., American Chemical Society 1988, ch. 2, pp. 7-32.
17. Gabriel, C.; Gabriel, S.; Grant, E.H.; Halstead, B.S.J.; Mingos, D.M.P. “Dielectric parameters relevant to microwave dielectric heating.” Chem. Soc. Rev. 1998, 27, pp. 213-23.
18. a) Internal Communication, CEM Corporation, Matthews, NC. b) This reaction was performed on a CEM Discover System. This microwave system is equipped with “hot keys”, which allow the user to change parameter values “on-the-fly”. One of these hot keys is for simultaneous controlled cooling. Once the reaction has reached its programmed temperature, the cooling option can be turned on with the hot key. This will ensure a constant, high power level. Use of the power hot key can then allow the user to increase the power level in small increments in order to maximize the amount of microwave energy being delivered to the reaction.
19. Welton, T. “Room-temperature ionic liquids. Solvents for synthesis and catalysis.” Chem. Rev. 1999, 99, pp. 2071-83.
20. a) Varma, R.S.; Namboodiri, V.V. “An expeditious solvent-free route to ionic liquids using microwaves.” J. Chem. Soc., Chem. Commun. 2001, 7, pp. 643-44. b) Varma, R.S.; Namboodiri, V.V. “Solvent-free preparation of ionic liquids using a household microwave oven.” Pure Appl. Chem. 2001, 73, pp. 1309-14.
21. Khadilkar, B.M.; Rebeiro, G.L. “Microwave assisted synthesis of room temperature ionic liquid precursor quaternary salts.” Fifth International Electronic Conference on Synthetic Organic Chemistry (ECSOC-5) 2001, E0020 (www.mdpi.net).
22. Leadbeater, N.E.; Torenius, H.M. “A study of the ionic liquid mediated microwave heating of organic solvents.” J. Org. Chem. 2002, 67, pp. 3145-48.
23. Fraga-Dubreuil, J.; Bazureau, J.P. “Rate accelerations of 1,3-dipolar cycloaddition reactions in ionic liquids.” Tetrahedron Lett. 2000, 41, pp. 7351-55.
24. Fraga-Dubreuil, J.; Bazureau, J.P. “Grafted ionic liquid-phase-supported synthesis of small organic molecules.” Tetrahedron Lett. 2001, 42, pp. 6097-100.
25. Fraga-Dubreuil, J.; Bazureau, J.P. “Ionic liquid-phase organic synthesis (IoLiPOS) methodology assisted by focused microwave technology: first results.” Fifth International Electronic Conference on Synthetic Organic Chemistry (ECSOC-5) 2001, E0011 (www.mdpi.net).
26. March, J. Advanced Organic Chemistry: Reactions, Mechanisms, and Structure, 4th Ed., John Wiley and Sons, 1992, ch. 10, p. 293, 340.