We use cookies to make your experience better. To comply with the new e-Privacy directive, we need to ask for your consent to set the cookies.
Theory of Microwave Heating for Organic Synthesis
Microwaves are a powerful, reliable energy source that may be adapted to many applications. Understanding the basic theory behind microwaves will provide the organic chemist with the right tools and knowledge to be able to effectively apply microwave energy to any synthetic route.
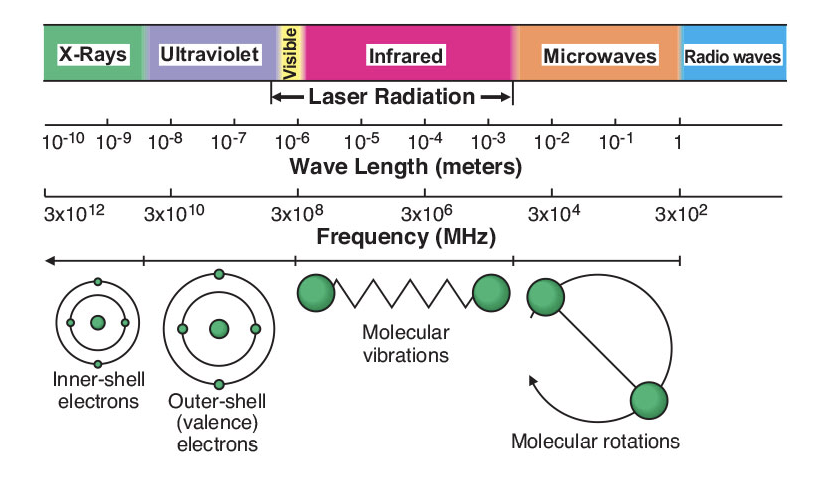
Figure 1: The electromagnetic spectrum
What are microwaves?
A microwave (Figure 1) is a form of electromagnetic energy that falls at the lower frequency end of the electromagnetic spectrum, and is defined in the 300 to about 300,000 megahertz (MHz) frequency range. Within this region of electromagnetic energy, only molecular rotation is affected, not molecular structure.1 Out of four available frequencies for industrial, scientific, or medical applications, 2450 MHz is preferred because it has the right penetration depth to interact with laboratory scale samples, and there are power sources available to generate microwaves at this frequency.
Microwave energy (Figure 2) consists of an electric field and a magnetic field, though only the electric field transfers energy to heat a substance.1 Magnetic field interactions do not normally occur in chemical synthesis. Microwaves move at the speed of light (300,000 km/sec). The energy in microwave photons (0.037 kcal/mole) is very low relative to the typical energy required to cleave molecular bonds (80-120 kcal/mole); thus, microwaves will not affect the structure of an organic molecule. In the excitation of molecules, the effect of microwave absorption is purely kinetic.
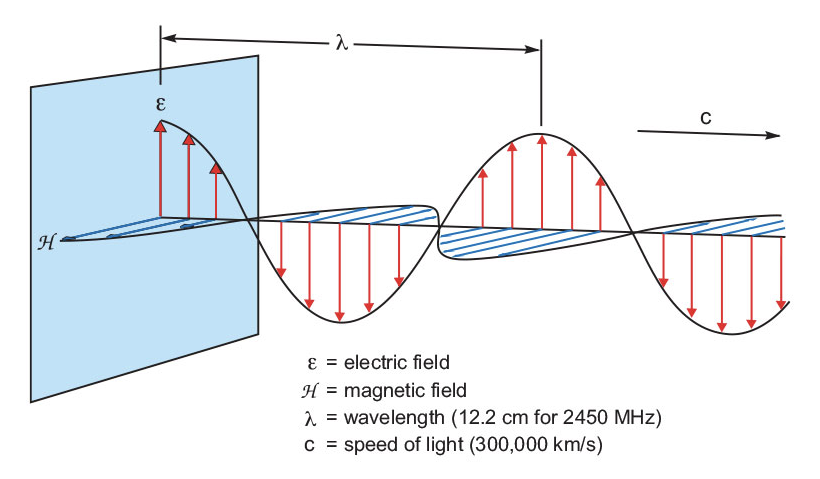
Figure 2: A microwave
How does a microwave heat a substance?
Traditionally, chemical synthesis has been achieved through conductive heating with an external heat source. Heat is driven into the substance, passing first through the walls of the vessel in order to reach the solvent and reactants (Figure 3). This is a slow and inefficient method for transferring energy into the system because it depends on the thermal conductivity of the various materials that must be penetrated. It results in the temperature of the vessel being higher than that of the reaction mixture inside until sufficient time has elapsed to allow the container and contents to attain thermal equilibrium. This process can take hours. Conductive heating also hinders the chemist’s control over the reaction. The heat source must physically be removed and cooling administered to reduce the internal bulk temperature.
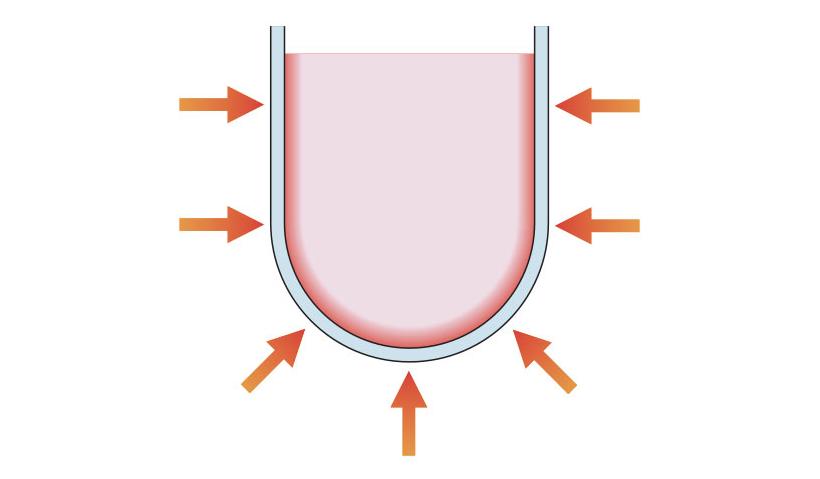
Figure 3: Schematic of sample heating by conduction.
Microwave heating, on the other hand, is a very different process. As shown in Figure 4, the microwaves couple directly with the molecules that are present in the reaction mixture, leading to a rapid rise in temperature. Because the process is not dependent upon the thermal conductivity of the vessel materials, the result is an instantaneous localized superheating of anything that will react to either dipole rotation or ionic conduction, the two fundamental mechanisms for transferring energy from microwaves to the substance being heated. Microwave heating also offers facile reaction control. It can be described as “instant on-instant off”. When the microwave energy is turned off, latent heat is all that remains.
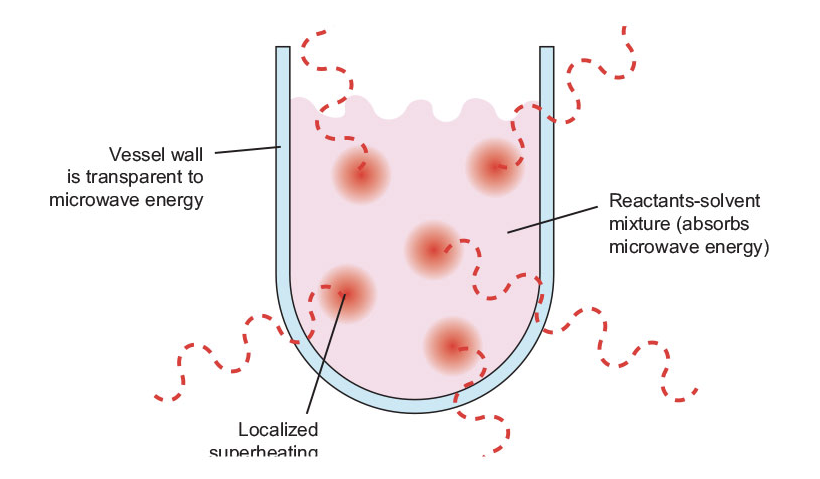
Figure 4: Schematic of sample heating by microwaves
Dipole rotation is an interaction in which polar molecules try to align themselves with the rapidly changing electric field of the microwave. The rotational motion of the molecule as it tries to orient itself with the field results in a transfer of energy. The coupling ability of this mechanism is related to the polarity of the molecules and their ability to align with the electric field. There are a number of factors that will ultimately determine the dipole rotation coupling efficiency; however, any polar species (solvent and/or substrate) that are present will encounter this mechanism of energy transfer.
The second way to transfer energy is ionic conduction, which results if there are free ions or ionic species present in the substance being heated. The electric field generates ionic motion as the molecules try to orient themselves to the rapidly changing field. This causes the instantaneous superheating previously described. The temperature of the substance also affects ionic conduction: as the temperature increases, the transfer of energy becomes more efficient.
How do microwaves increase reaction rates?
In a typical reaction coordinate (Figure 5), the process begins with reactants (A and B), which have a certain energy level (ER). In order to complete the transformation, these reactants must collide in the correct geometrical orientation to become activated to a higher-level transition state (ETS). The difference between these energy levels is the activation energy (Ea) required to reach this higher state (ETS – ER = Ea). The activation energy is the energy that the system must absorb from its environment in order to react. Once enough energy is absorbed, the reactants quickly react and return to a lower energy state (EP) — the products of the reaction (A-B). Microwave irradiation does not affect the activation energy, but provides the momentum to overcome this barrier and complete the reaction more quickly than conventional heating methods.
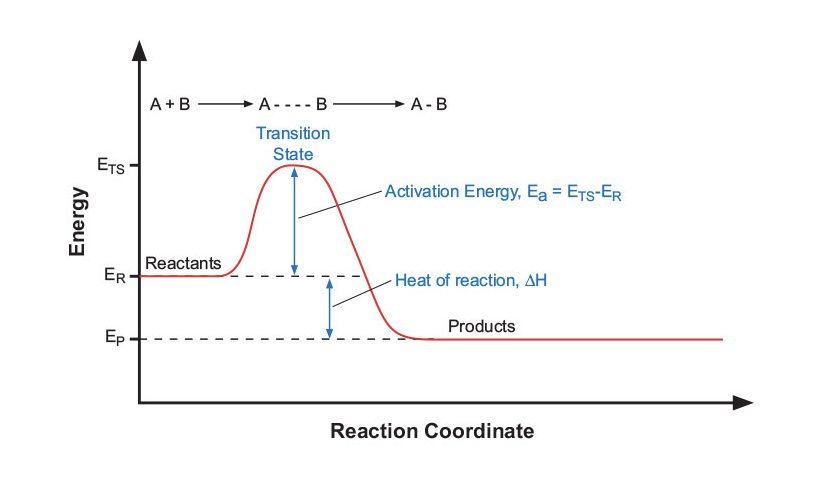
Figure 5: Reaction coordinate
In general drug discovery and development work, for example, an activation energy value may be 50 kcal/mole. A representative process would involve 30 mg of each reactant, leading to approximately 30 mg of product(s) with an average molecular weight of 300 g/mole (300-350 is characteristic of present drug compounds). According to the calculations in Figure 6, five calories of energy are required for the full transformation to occur. Commercial microwave hardware available today typically delivers 300 W of power. Translated into calories, this indicates that 72 cal/sec of energy are available from the 300 W of microwave power, assuming a 100% efficiency in microwave heating. Clearly, the amount of microwave energy being introduced to the system is very large relative to the energy needed to achieve the required activation energy. It is this phenomenon that contributes to the increased reaction speeds and higher yields that occur in microwave chemistry.
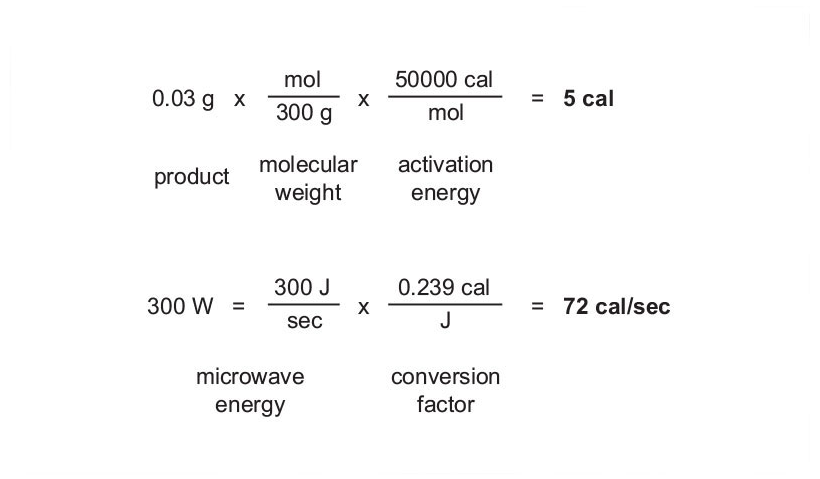
Figure 6: Microwave energy vs. required activation energy
One of the most important aspects of microwave energy is the rate at which it heats (Figure 7). Microwaves will transfer energy in 10-9 seconds with each cycle of electromagnetic energy. The kinetic molecular relaxation from this energy is approximately 10-5 seconds. This means that the energy transfers faster than the molecules can relax, which results in the non-equilibrium condition and high instantaneous temperatures that affect the kinetics of the system. This, in turn, enhances the reaction rate, as well as the product yields. In addition, the lifetime of activated complexes are approximately 10-13 seconds, and thus, are much shorter lived than the rate at which energy is transferred with microwaves. Activated complexes do not normally exist long enough to have an opportunity to absorb microwave energy. However, there are a number of resonance-stabilized intermediates that are much longer lived. Many of these have lifetimes longer than 10-9 seconds, so the opportunity exists in certain chemical reactions, for intermediates generated in this approximate time frame, to couple directly with the microwave and be further enhanced. Most intermediates are highly polar species and many of them are even ionic in character, making them excellent candidates for microwave energy transfer.
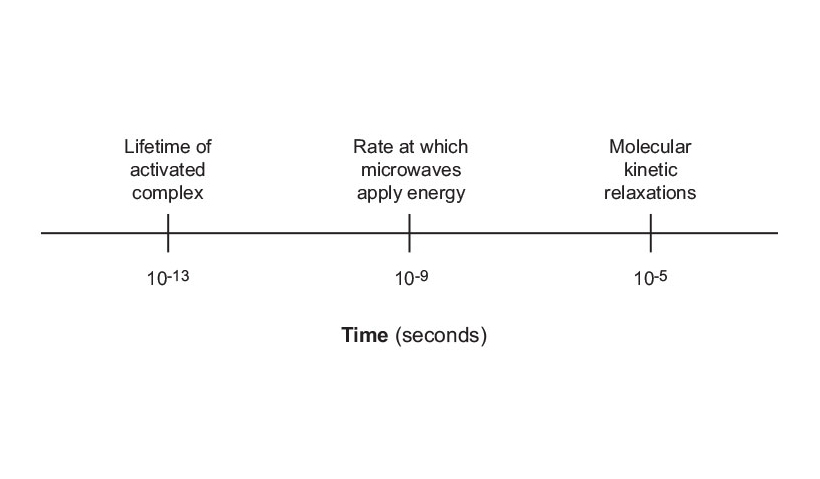
Figure 7: Speed of microwave heating
Based on the Arrhenius reaction rate equation (k = Ae-Ea/RT), the reaction rate constant is dependent on two factors: the frequency of collisions between molecules that have the correct geometry for a reaction to occur (A) and the fraction of those molecules that have the minimum energy required to overcome the activation energy barrier (e-Ea/RT). There has been some speculation that microwaves affect the orientation of the molecular collisions and the activation energy, but there is no evidence that supports either of these ideas. 5,6 Microwaves do not influence the orientation of those collisions, nor the activation energy. Activation energies remain constant for each particular reaction. However, microwave energy will affect the temperature parameter in this equation. An increase in temperature causes molecules to move about more rapidly, which leads to a greater number of more energetic collisions. This occurs much faster with microwave energy, due to the high instantaneous heating of the substance(s) above the normal bulk temperature, and is the primary factor for the observed rate enhancements.
Arrhenius reaction rate equation
(k = Ae-Ea/RT)
It should also be obvious that the level of instantaneous heating will be dependent on the amount of microwave energy that is used to irradiate the reactants. The higher the level of microwave energy, the higher the instantaneous temperature will be relative to the bulk temperature. One method for increasing the microwave energy that is delivered is to use simultaneous cooling during the microwave irradiation. This allows a higher level of microwave power to be directly administered, but will prevent overheating by continuously removing latent heat. This technique has proven to be very effective in further enhancing of reaction rates and will be discussed in greater detail throughout the book.
Based on experimental data from numerous works that have been performed over the last ten years, chemists have found that microwave-enhanced chemical reaction rates can be faster than those of conventional heating methods by as much as 1,000-fold.3,4,7-15 Assuming the standard first order rate law (rate = k[A]), the Arrhenius rate equation (k = Ae-Ea/RT) was used to calculate the instantaneous temperatures required to get the reaction enhancements shown in Figure 8. The assumption was a desired reaction bulk temperature of 150 °C and an activation energy of 50 kcal/mole for the transformation. For a 10-fold rate increase, it was determined that a temperature enhancement of only 17 °C would be needed relative to a bulk temperature of 150 °C. Microwave energy can provide that temperature increase instantly. Likewise, for a 100-fold rate increase, the temperature would have to reach 185 °C and would require approximately a 35 °C increase over the bulk temperature. A 1000-fold enhancement would need a 56 °C increase. These instantaneous temperatures are very consistent with the temperatures that would be expected in a microwave system and are directly responsible for the reaction rate and yield enhancements.
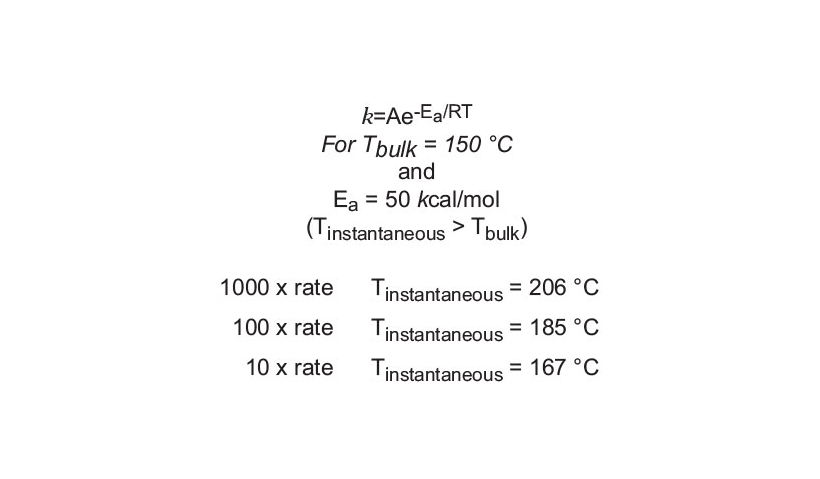
Figure 8: Enhanced reaction rates
What type of reactions are effected by microwave heating?
There are two main types of chemical reactions, kinetic and thermodynamic (Figure 9). Chemical reactions driven by conventional heating are more likely to perform under kinetic control (Reaction 1, Figure 9). These reactions usually require only mild conditions. A resonance-stabilized intermediate will take the easiest path — one with the lowest activation energy — to its products. Alternatively, thermodynamically controlled reactions have higher activation energies and require harsh conditions to complete (Reaction 2, Figure 9). In microwave driven reactions, the molecules are provided powerful instantaneous energy, which allows them to reach these higher activation energy levels and leads to the thermodynamic product. This mechanism is a probable explanation for some of the work that has been done recently on highly diastereoselective syntheses, which were generated using microwave irradiation versus conventional heating. 16
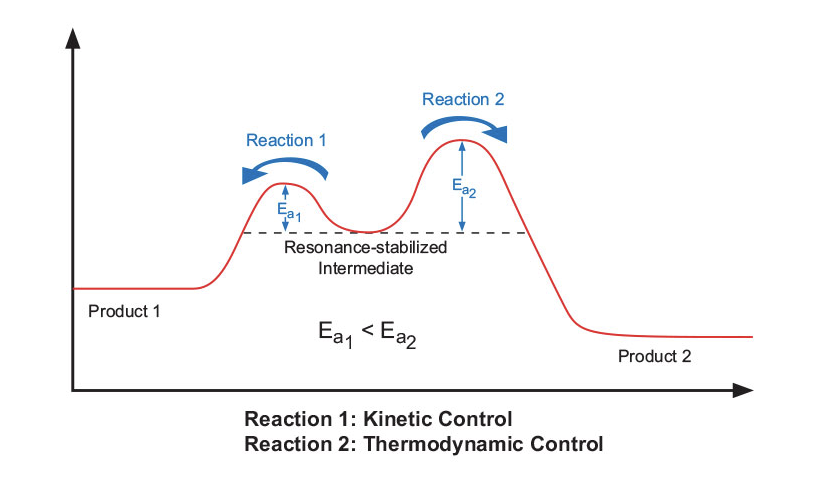
Figure 9: Kinetically vs. thermodynamically controlled reactions
Clearly, microwave heating is extremely useful in slower reactions where high activation energies are required to do various transformations. Empirically, the activation energy parameter expresses the temperature dependence of the rate constant. A small Ea corresponds to a rate constant that does not increase rapidly with temperature, whereas a reaction with strong temperature dependence has a large Ea. With the elevated molecular energy generated by the transfer of microwave energy, reactions that required many hours or even days to complete have been accomplished in minutes. It is also possible to use non-polar solvents to actually reduce bulk heating and directly energize the molecule. The solvent acts as a heat sink to pull thermal heat away from the reactants. The use of non-polar solvents in this manner will open opportunities to perform temperature-sensitive reactions that were not possible with conventional heating. This will be discussed in greater detail in Chapter 2. Microwave-enhanced synthesis greatly expands the options organic chemists have in their search for new compounds. Drug discovery can be taken to new heights as chemists explore the depths of their creativity.
Instruments
1. Neas, E.D.; Collins, M.J. Introduction to Microwave Sample Preparation Theory and Practice, Kingston, H.M.; Jassie, L.B., Eds., American Chemical Society 1988, ch. 2, pp. 7-32.
3. Giguere, R.J.; Bray, T.L.; Duncan, S.M.; Majetich, G. Application of commercial microwave ovens to organic synthesis. Tetrahedron Lett. 1986, 27, pp. 4945-48.
4. Gedye, R.; Smith, F.; Westaway, K.; Ali, H.; Baldisera, L.; Laberge, L.; Rousell, J. The use of microwave ovens for rapid organic synthesis. Tetrahedron Lett. 1986, 27, pp. 279-82.
5. Jun, C.H.; Chung, J.H.; Lee, D.Y.; Loupy, A.; Chatti, S. “Solvent-free chelation-assisted intermolecular hydro-acylation: effect of microwave irradiation in the synthesis of ketone from aldehyde and 1-alkene by Rh(I) complex.” Tetrahedron Lett. 2001, 42, pp. 4803-05.
6. Loupy, A.; Perreus, L.; Liagre, M.; Burle, K.; Moneuse, M. “Reactivity and selectivity under microwaves in organic chemistry. Relation with medium effects and reaction mechanisms.” Pure Appl. Chem. 2001, 73, pp. 161-66.
7. Mingos, D.M.P.; Baghurst, D.R. “Applications of microwave dielectric heating effects to synthetic problems in chemistry.” Chem. Soc. Rev. 1991, 20, pp. 1-47.
8. Loupy, A.; Petit, A.; Hamelin, J.; Texier-Boullet, F.; Jacquault, P.; Mathe, D. “New solvent-free organic synthesis using focused microwaves.” Synthesis 1998, 9, pp. 1213-34.
9. Loupy, A. “Microwaves in organic synthesis: a clean and high-performance methodology.” Spectra Anal. 1993, 22, p. 175.
10. Majetich, G.; Hicks, R. “Applications of microwave-accelerated organic synthesis.” Radiat. Phys. Chem. 1995, 45, pp. 567-79.
11. Bose, A.K.; Manhas, M.S.; Ghosh, M.; Shah, M.; Raju, V.S.; Bari, S.S.; Newaz, S.N.; Banik, B.K.; Chaudhary, A.G.; Barakat, K.J. “Microwave-induced organic reaction enhancement chemistry. 2. Simplified techniques.” J. Org. Chem. 1991, 56, pp. 6968-70.
12. Johannsson, H. “A solution to the bottleneck in drug discovery.” Am. Laboratory 2001, 33, pp. 28-32.
13. Larhed, M.; Hallberg, A. “Microwave-assisted high-speed chemistry: a new technique in drug discovery.” Drug Discovery Today 2001, 6, pp. 406-16.
14. Strauss, C.R.; Trainor, R.W. “Developments in microwave-assisted organic chemistry.” Aust. J. Chem. 1995, 48, pp. 1665-92.
15. Langa, F.; De La Cruz, P.; De La Hoz, A.; Diaz-Ortiz, A.; Diez-Barra, E. “Microwave irradiation: more than just a method for accelerating reactions.” Contemp. Org. Synth. 1997, 4, pp. 373-86.
16. Kuhnert, N.; Danks, T.N. “Highly diastereoselective synthesis of 1,3-ozazolidines under thermodynamic control using focused microwave irradiation under solvent-free conditions.” Green Chem. 2001, 3, pp. 68-70.